Chapter 1
History of Perovskites: Where Did Perovskite Solar Cells Come From?
The first perovskite material discovered was the compound Calcium Titanate (CaTiO3), also known as the mineral Perovskite. It was named by its discoverer Gustav Rose in 1839, in honour of noted Russian mineralogist Lev Aleksevich von Perovski.Â
Later, in 1892, the first synthesis of a cesium lead halide perovskite material in history was successfully performed. This is important because it is the basis for the chemical composition of modern perovskite solar cells (PSC). However, it wasn’t until 1926, almost 90 years later, that the perovskite crystal structure was theoretically described. It took another 19 years for that theory to be confirmed via X-ray crystallographic investigation of a similar material, Barium Titanate, in 1945.Â
For decades after 1957, perovskite materials found their way into more electric and electrochemical devices and even perovskite-structured superconducting materials. But the next step toward our modern focus in the field of perovskites was not until 1978 with the synthesis of the first organic-inorganic lead halide perovskite material. This new semiconductive material exhibited several interesting optical and electronic properties such as a very high absorption coefficient and high relative permittivity.Â
However, the use of an organic lead-halide perovskite as the central component of a solar cell would not happen until 2009 with an experiment meant to attempt to replace low voltage ruthenium-based inks in dye-sensitized solar cells with higher voltage perovskite-based ink.
Perovskite materials are flexible in purpose and ability, able to perform varied tasks depending on their formulation. Below, we’ll discuss the reasons for that flexibility, and many of the ensuing outcomes.
What Is The Structure Of Perovskites And What Makes It Special?
What about the perovskite material made them worthy of so much scientific investigation and application? Compared to other, more traditional solar cell technologies such as silicon or cadmium telluride, perovskites have incredible flexibility in the materials that comprise their structure. Let’s first discuss the nature and reasons for this flexibility before we get into the important implications this has for perovskite behaviour.
Composition And Common Materials Of Perovskite Solar Cells
A perovskite is a three-component system consisting of a central A atom/molecule surrounded by 8 ligands. Each ligand is a B atom surrounded by 6 X atoms in an octahedral or square pyramidal geometry. Due to how atoms are shared between unit cells of a material, this results in a generalized chemical formula of ABX3 for a traditional perovskite material such as the original mineral CaTiO3 (an oxide perovskite).
However, oxide perovskites are not the type of material currently used in photovoltaic (PV) solar cells. Instead, perovskite solar cells primarily use organic-inorganic halides with the most common being methylammonium lead iodide (MAPbI3). However, just because it is the most common does not mean it is the only viable composition. As we’ll discuss later, material researchers continue to find new and interesting ways of making perovskites for the best outcomes.
Below, you can find a table of known alternative component atoms/molecules for their respective positions in the ABX3Â structure of perovskite.
B position element |
X position element |
|
Methyl Ammonium |
Lead |
Iodine |
Formamidinium |
Tin |
Bromine |
Cesium |
Germanium |
Chlorine |
Rubidium |
Bismuth |
 |
Guanidinium |
Antimony |
 |
Butyl Ammonium (n, t, or iso) |
 |  |
Ethyl Ammonium |
 |  |
Benzylammonium |
 |  |
Acetamidinium |
 |  |
Imidazolium |
 |  |
Dimethyl Ammonium |
 |  |
n-Propyl Ammonium |
 |  |
 Reference: https://www.ossila.com/en-ca/collections/perovskite-precursor-materialsÂ
What Makes The ABX3Â Perovskite Structure So Special?
With the number of possible components shown above, you can synthesize over 200 different perovskite crystals through varying component combinations without considering fractional mixtures or potential dopants. What’s important to consider about these component possibilities is that they are not all identical in size or structure and that can have interesting effects on the perovskites themselves due to how crystal lattice stresses can affect and alter electronic and even chemical properties.
As we described above, the basic form of perovskite is a cubic crystal comprised of a central component atom/molecule A adjacent to 8 ligands of B and X components. Depending on what those components are, and their relative sizes to each other, it is possible to stress the crystal out of its normal shape into several other alternate perovskite structures.
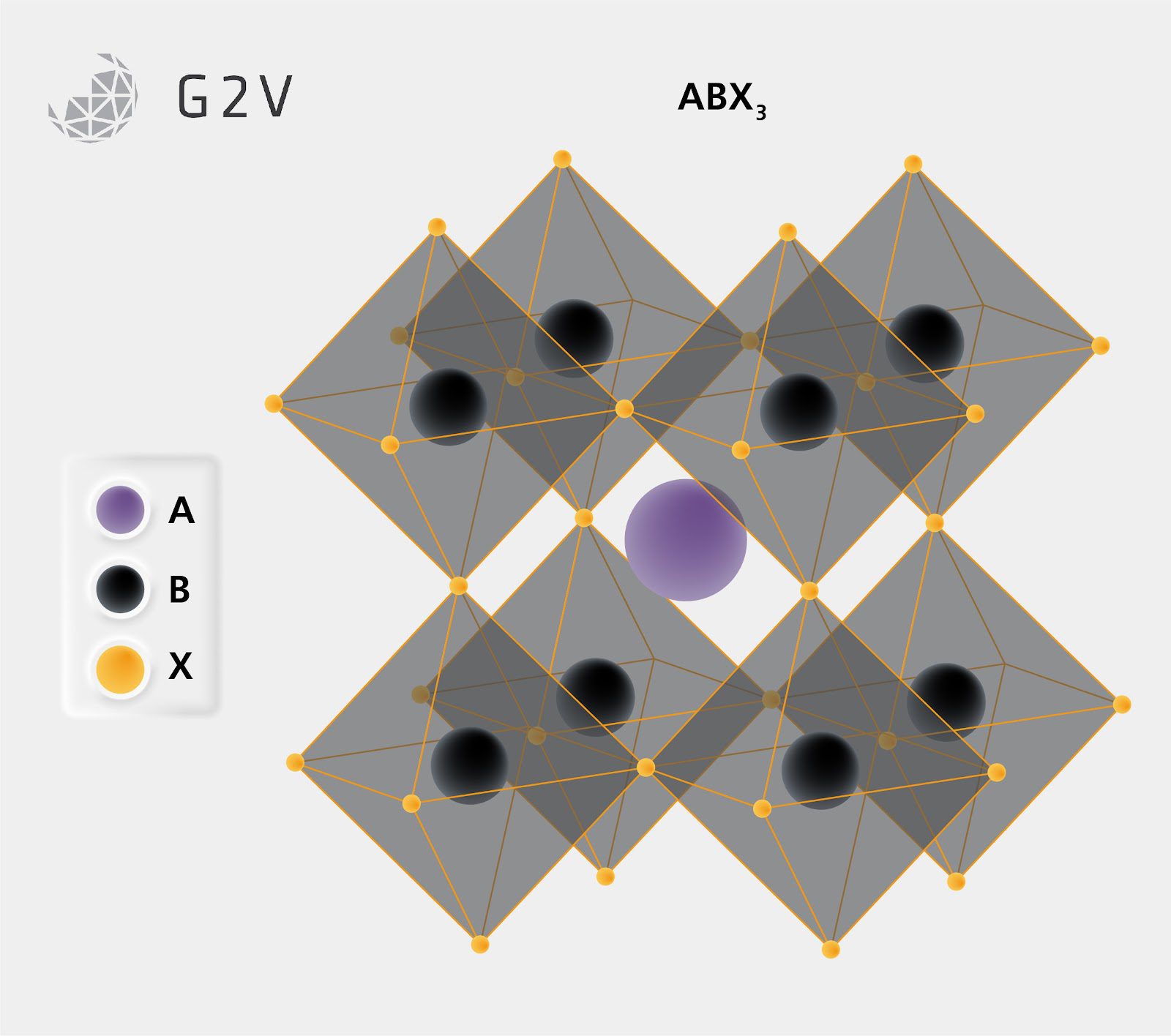
Stressing a crystal by altering a crystal’s components is a well-accepted method of modifying both physical and electronic properties. One example is the alteration or substitution of crystal components to obtain different piezoelectric behaviour.
With so many different possible components of such varying sizes and molecular structures, what novel features can be built into perovskite solar cells?
Changing the X component among the three common halides without changing the other components can cause rotation of the octahedral structures, enforcing a lattice change from cubic to tetragonal by extending one axis of the perovskite crystal compared to the other two as a method to reduce lattice strain, or vice versa depending on substitution. There is a known correlation between crystal structure and semiconductor bandgap, so being able to influence that with a simple chemical substitution is very useful.
Altering the B component can also create what is known as a double perovskite structure if B is fractionally replaced, which allows for even more freedom and possibility in how component replacement can impact the desired structure while still providing useful or novel properties such as removing lead from the structure or producing ferroelectric effects.
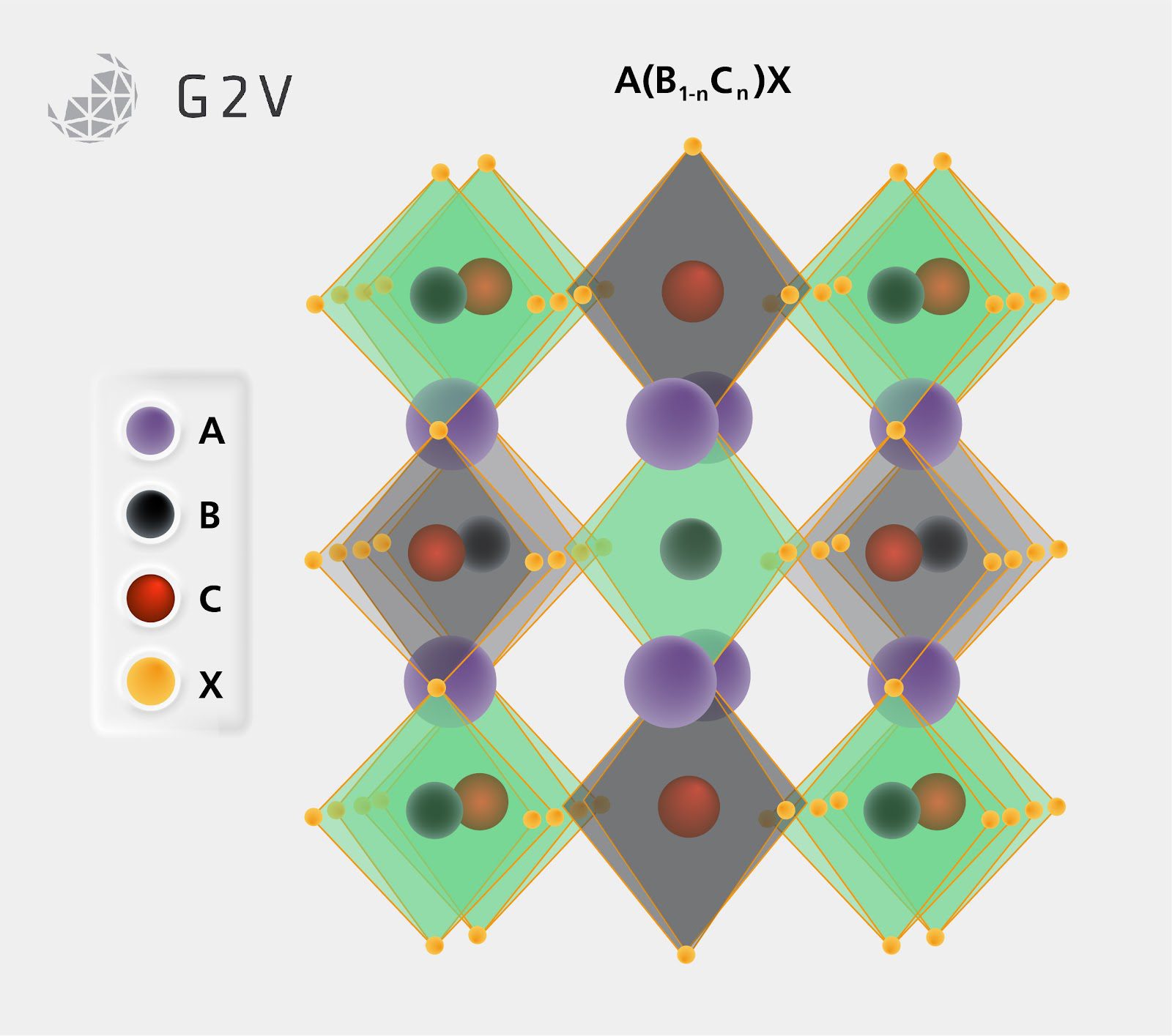
Changing the A component can also shift a perovskite’s structure in fairly significant ways depending on the size of the molecule. If the A component’s diameter is too large compared to the surrounding BX ligands, the larger A components apply stress on the crystal to make room for themselves. This can result in a crystallographic shift from the common 3-dimensional perovskite ABX3 structure to what is known as the Ruddleson-Popper or 2D perovskite structure. This structure consists of 2-dimensional layers of perovskite BX ligands separated by a shifted pattern of A component materials.
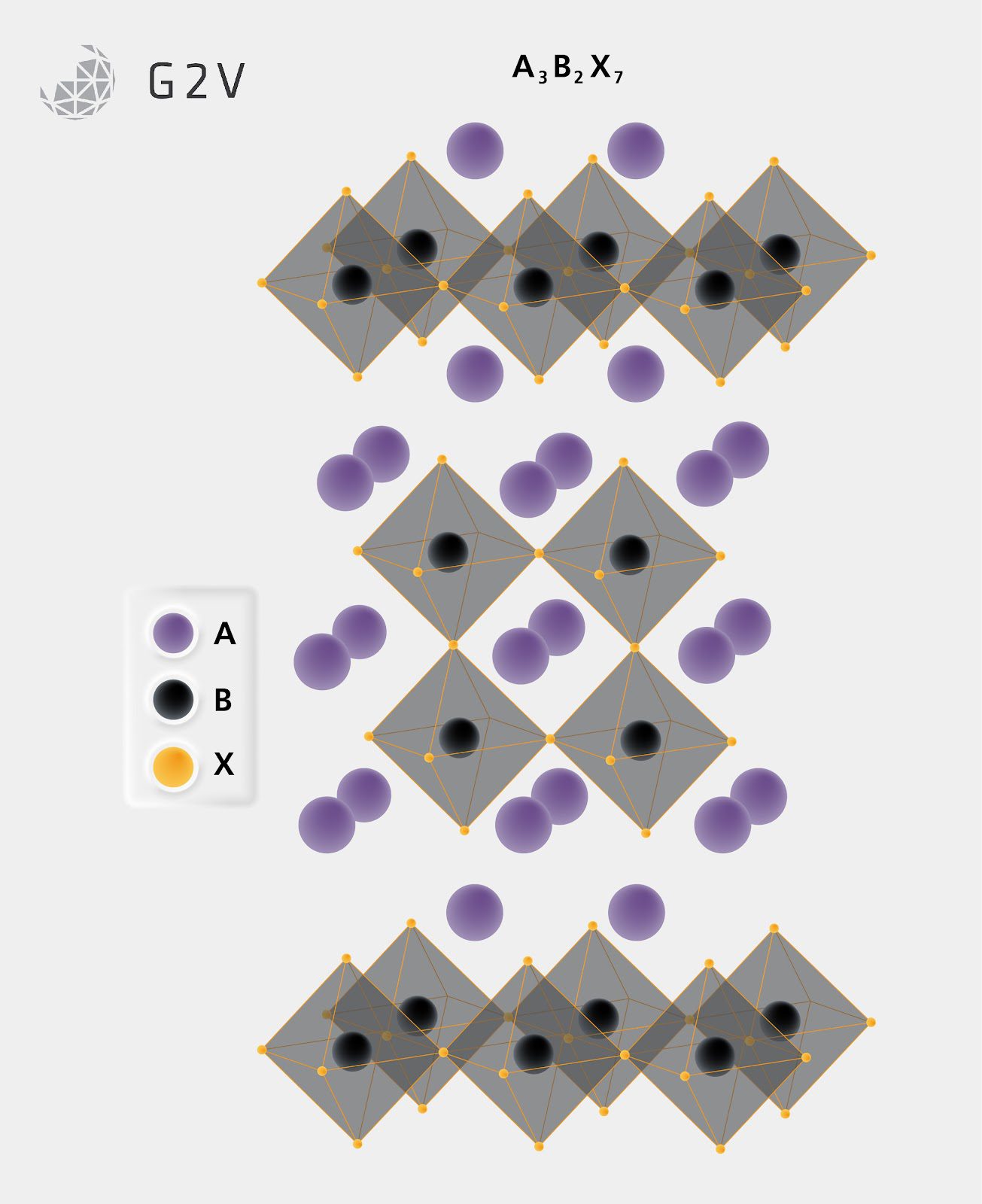
The stress from varying a perovskite’s A component can also alter the chemical composition from ABX3 to A2BX4 or even the A3B2X7 ratio. This occurs by the internal stress of the perovskite cubic crystal being too large to allow the BX ligands to connect as normal and allow other A components to insert themselves into new positions, altering the crystal make-up so that these 2D structures are the most energetically favourable.
Shifting the dimensionality of the crystal can directly impact the electronic properties. Due to higher internal stress on the crystal, a 2D structure can act as a barrier to the formation of defects in the crystal, which is very useful for preventing a common source of reduced power conversion efficiency (PCE) and slowing down the degradation of the cell. With so many options for components, and an understanding of how replacing, substituting, or mixing can alter the structure and thus the electronic landscape of the crystal structure, perovskites can offer a flexible platform for tuning solar cell properties for different applications.
In crystals, defects form and move based on the energy of the bonds between the components. In a single crystal, defect movement is easier due to a crystal being the lowest local energy state for the material meaning it takes less energy for the defect to move through it compared to a more disordered and thus higher energy structure. Perovskites’ ability to be built from mixed and matched components makes it possible to build barriers within the crystal out of the different structures and components so that defects have a harder time forming and can be controlled in their movements according to a researcher’s desires.
Understanding the atomic construction of perovskite crystals is essential for producing high-quality solar cells, however, it is also necessary to understand how these materials can be reliably made at scale if they are to be successfully deployed in solar modules. We’ll discuss perovskite fabrication techniques in the next chapter, Perovskite Cell Manufacturing.
Next Chapter 2: How Are Perovskite Solar Cells Made?
Looking for a cheat sheet with all of the key concepts in this article? Check out the one-page infographic below.